The Development of Precision Robotic Photometry
Robotic Telescopes
ASP Conference Series, Vol. 79, 1995
Gregory W. Henry and Joel A. Eaton (eds.)
Gregory W. Henry
Center of Excellence in Information Systems, Tennessee State University
330 10th Ave. North, Nashville, TN 37203
Abstract. A robotic telescope development program has been underway at Tennessee State University for the past several years, driven by the desire to measure smaller and smaller stellar luminosity changes. The goal of this program has been to increase the precision and efficiency of photometric observations from robotic telescopes as well as to automate the reduction and archiving of the resulting data. Through the use of larger telescopes, precision photometers, new observing strategies, and detailed quality control monitoring, we have succeeded in improving the precision of robotic telescope photometry by an order of magnitude. The internal precision of observations with our newest telescopes matches the expected photon and scintillation noise limits, and the rigorous quality control made possible by automation results in a level of external precision unmatched with traditional manual telescopes.
1. Introduction
For the past several years, observational programs at Tennessee State University in chromospherically active stars, solar-type stars, solar duplicates, and chromospheric structure have made it clear that our understanding of stellar magnetism is severely constrained by the lack of sufficient observational data. We have attempted to alleviate this situation somewhat through the development of automated photometry. We are also planning for the development of automated spectroscopy (see Eaton, "The Rationale for an Automatic Spectroscopic Telescope," this volume).
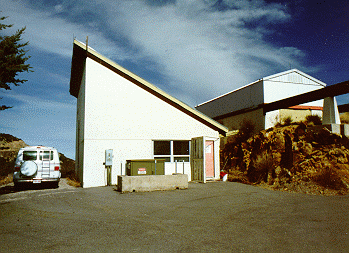
Figure 1. The Fairborn Observatory APT site on Mt. Hopkins. On the right is the roll-off shelter housing 8 APT's. On the left is the control building containing computer systems, workshops, and other support facilities.
This paper describes the operation of four automatic photoelectric telescopes (APT's) in order to document the gains made in performance of these systems in recent years. All four telescopes, along with others, are located under a single roll-off-roof shelter at the Fairborn Observatory (a non-profit organization headed by Lou Boyd for the development and operation of robotic telescopes) on Mt. Hopkins (Figure 1; Eaton, Figure 1, this volume). These telescopes have brought revolutionary improvements to our long-term photometric monitoring programs (see Henry, "The Fairborn/TSU Robotic Telescope Operations Model," this volume). We have acquired over 150,000 sets of differential observations with these telescopes, and, with collaborators at various institutions, have published over 100 scientific papers over the past six years dealing with APT results.
2. The Fairborn 10-inch APT
The Fairborn 10-inch APT (Figure 2) was the first of (currently) eight APT's to go into operation on Mt. Hopkins. The tube assembly and optics are from Meade Instruments, the mount is from DFM Engineering, and the SSP-3 photometer (with an ambient temperature photodiode detector) is from Optec. Since early 1986, this telescope has collected over 49,000 group observations of variable stars through Johnson V, R, and I filters in a joint observing program with the Harvard-Smithsonian Center for Astrophysics. The observations are conducted in a fixed group sequence: K,S,C,V,C,V,C,V,C,S,K where K is a check star, S is a sky position, C is a comparison star, and V is the variable star. We are restricted to this fixed sequence by the telescope's primitive (compared to more recent APT's) control system that cannot be programmed to handle other kinds of observing sequences.
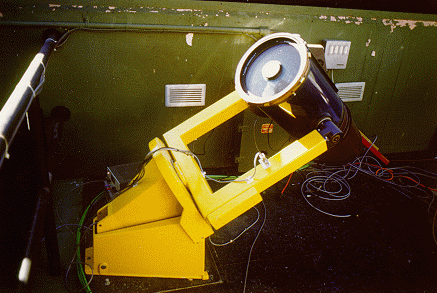
Figure 2. The Fairborn 10-inch APT. This telescope is being used by Tennessee State University and the Harvard-Smithsonian Center for Astrophysics to monitor a variety of variable stars, especially semi-regular variables.
The precision of the data acquired with this telescope is limited by several factors. (1) The small aperture of the telescope causes significant scintillation noise. Young (1974) provides a method of estimating the scintillation noise of an observation as a function of telescope aperture, observatory altitude, integration time, and air mass. In the case of the 10-inch telescope on Mt. Hopkins (7800 ft. above sea level), the scintillation noise for a group mean taken at a median airmass of 1.35 with our 10 second integration times would be about 0.0035 mag, after correction for a factor of two error in Young's original formula (Young 1991). (2) The internal precision (i.e. the repeatability of successive differential magnitude measurements within a group) is also limited by imperfections in the centering process. The telescope centers stars in the focal-plane diaphragm of the photometer by taking counts with the photometer in four overlapping regions of the sky centered around the presumed position of the star (Boyd, Genet, & Hall 1984). The counts in these four regions are compared, the direction to the true star position derived, and the telescope moved in that direction. This process is repeated until the count rates in all four regions are the same, indicating that the telescope has properly centered the star. The centering accuracy is only one-fourth to one-third of the diaphragm diameter. (3) Wear in the telescope's worm-gear drive systems resulting from heavy use in the automated observing mode places additional limits on the internal precision. This wear makes it difficult for the preloaded drives to resist motion due to wind loading or slight imperfections in telescope balance and so aggravates the already marginal centering accuracy. (4) The primitive control system precludes the possibility of measuring standard stars to determine nightly extinction coefficients. Therefore, the external precision (i.e. the repeatability of night-to-night group mean differential magnitudes of constant star pairs) is degraded because the data must be reduced with long-term mean extinction coefficients determined on occasional nights of standard star observations. (5) The photometer undergoes seasonal and night-to-night changes in color sensitivity due to ambient temperature changes. Again, because nightly standard stars cannot be observed, the data are reduced with mean transformation coefficients. Figure 3 shows the effect of seasonal temperature variations on Johnson V transformation coefficients determined occasionally over a two and one-half year period.
Because the 10-inch APT (as well as our other APT's) has no way of knowing the quality of a night in real time, it collects data as long as it can find stars. Therefore, a way must be found to eliminate ex post facto data that were taken in non-photometric conditions. The stars we typically observe with APT's are not expected to vary significantly over the few minutes required to obtain a group observation. Therefore, we use the internal precision of a group observation as measured by the standard deviation of the group mean differential magnitudes as our cloud filter. In good photometric conditions, these group mean standard deviations average about 0.005 to 0.007 mag. Thus, to filter the data at the 3-sigma level, we eliminate any group mean with a standard deviation larger than 0.02 mag. This cloud filter works extremely well and few errant points survive the filtering process. The fact that our internal errors are considerably larger than the scintillation noise estimated above, even for bright stars where photon noise is 0.001 mag or less, indicates that a substantial fraction of these errors is due to coarse centering of stars in the diaphragm.
To determine the external precision of data from the Fairborn-10, we measure pairs of constant stars each night. Figure 4 displays data on the constant pair HD 125451 / HD 124570 from one observing season. Each point in the figure represents one nightly group mean differential magnitude in the V band, and the 0.010 mag standard deviation of the nightly observations from the seasonal mean represents the external precision. Essentially the same precision is obtained in the R and I filters. This matches the photometric precision documented by Young et al. (1991) for the Phoenix-10 APT which began operations more than 10 years ago in Boyd's back yard and was subsequently moved to Mt. Hopkins in 1986.
This 1% precision is adequate to produce beautiful long-term lightcurves of semi-regular variable stars with amplitudes of a few tenths of a magnitude. Figure 5 shows eight seasons of V data on RX Leporis. These data and data from nine other semi-regular variables were analyzed recently by Cristian et al. (1995). Several of the stars, including RX Leporis, show two or three distinct timescales of pulsation, so this type of long-term monitoring is just beginning to reveal the true pulsational characteristics of these stars.
3. The Vanderbilt/Tennessee State University 16-inch APT
The VU/TSU 16-inch APT (Figure 6) went into operation in November 1987 to study chromospherically active stars, which vary typically by only 0.1 mag or so. DFM Engineering manufactured the mechanical components of the telescope. Paul Jones of Star Instruments produced the optics, and Lou Boyd at Fairborn designed and built the control system and photometer, which uses an EMI 9924B photomultiplier tube. This joint Vanderbilt/Tennessee State collaboration has produced over 72,000 Johnson B and V group observations of over 200 stars. Some of the early problems encountered in the operation of the 16-inch APT have been described in Hall & Henry (1993).
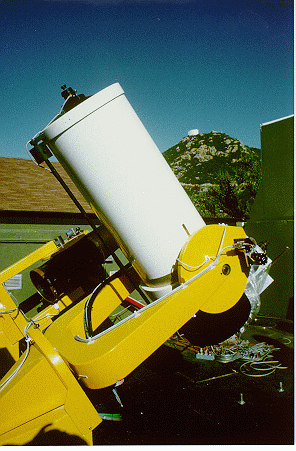
Figure 6. The Vanderbilt/Tennessee State 16-inch APT. The precision photometer built by Boyd is contained in the dark tub on the tail of the telescope.
During its first three years, the 16-inch was operated in a mode identical to the Fairborn-10 described above. At the end of the third year, the original worm-gear drives on both axes were replaced with micro-stepping belt-drive systems designed by Boyd. These belt-drive systems provide better resistance to wind loading, are free of backlash, allow much faster slewing of the telescope, and eliminate the mechanical wear associated with the worm drives. At the time the drives were upgraded, a new control system was installed that is capable of running the Automatic Telescope Instruction Set (ATIS) (Genet & Hayes 1989) developed at Fairborn. With ATIS, we have the ability to modify our differential observing sequences and integration times as needed, although we continue to use primarily the standard sequence described above for the Fairborn-10. After four years of operation, the original photometer was replaced by a new precision photometer designed and built by Boyd. This new photometer features a CCD camera for finding and centering stars in the diaphragm. Centering times improved from around 10 to 15 seconds with the old centering routine (described above) to about two seconds with the CCD camera. Centering accuracy also improved to approximately two arcseconds. Once a star is properly centered in the diaphragm, a rotating mirror moves out of the light path to admit light to the photomultiplier. Filtered and dried air isconstantly circulated inside the sealed enclosure of the photometer to control dust and humidity. Accurate temperature control is provided by a combination of thermoelectric coolers and circulating coolant that stabilize the enclosed photomultiplier, filters, and electronics to within a degree or so Fahrenheit. Two rotating filter wheels and one rotating diaphragm wheel provide a large selection of color and neutral density filters and diaphragm sizes. The improved efficiency of the belt drives and CCD centering allowed us to place approximately 50% more program stars on the observing menu than we were able to observe previously.
Since the precision photometer went into operation in early 1992, we have used ATIS to schedule nightly observations of standard stars selected automatically from a master list of standards covering the observable sky. A least-squares fit to each night's standard star observations produces a simultaneous solution for the nightly extinction coefficients, transformation coefficients, and zero points. These nightly coefficients are accepted and saved only if a sufficient number of standard star observations are made over sufficient ranges of airmass and color index, and if the rms of the all-sky solution is less than 0.03 mag. The differential reductions of the program star data then proceed with the nightly extinction coefficients, if available, or a running average of the three most recent nightly extinction coefficients if the standard star solution was not satisfactory. Only long-term averages of the nightly transformation coefficients are used to convert our differential magnitudes to the standard system. The internal precision, i.e. the average nightly standard error of the group means, now averages between 0.0025 and 0.0035 mag on good nights, so only observations that survive a 0.01 mag (3-sigma) cloud filter are saved. All reductions occur each morning under control of automated routines developed for a PC.
Nightly transformation coefficients for the past two years are plotted in Figure 7. Gaps in the data occur each year when the site is shut down for protection against intense lightning storms prevalent in July and August. Compared to results in Figure 3 from the Fairborn-10, it is obvious that careful temperature control of the precision photometer has eliminated seasonal variations in the transformation coefficients.
Nightly extinction coefficients in V are shown in Figure 8. This record begins approximately six months after the gigantic eruption of the Mt. Pinatubo volcano in the Philippines in June 1991. When the resulting dust cloud reached southern Arizona, extinction coefficients tripled. Since then, the usual seasonal variations inextinction have been superimposed on a gradual decline due to the dissipation of dust and high-altitude aerosols from Pinatubo. Extinction finally returned to normal levels in late 1994.
Nightly zero points (in magnitudes) are plotted in Figure 9. While not used in the differential reductions, these zero points are extremely useful for monitoring the stability of the system and for deciding when to clean the optics. A linear decrease with time over several months is generally seen as dirt accumulates on the mirrors and photometer entrance window, followed by a jump when the optics are washed.
Since the precision photometer was installed, the internal precision of the 16-inch APT typically ranges from 0.0025 to 0.0035 mag. Young's scintillation model predicts 0.0025 mag of scintillation noise for a typical group mean from the 16-inch. This, combined with a photon noise of 0.001 mag for a typical group observation, yields an expected internal precision of 0.0027 mag, in good agreement with what we actually observe. Therefore, the internal precision our observations is no longer limited by centering or tracking problems, but can be completely accounted for by scintillation and photon noise.
An eight-year history of the external precision from the 16-inch is written in the observations of the constant red/blue pair 27/28 Leo Minoris (Figure 10). The first four seasons of data were taken with the original, ambient-temperature photometer. The external precision increased over the first three years from 0.009 mag to 0.016 mag due to wear in the worm-gear drives. Precision in the fourth season improved to 0.008 mag with the installation of the new belt-drive systems, but the original photometer and crude centering algorithm were still in use. The precision photometer was installed prior to the fifth season. The improved CCD centering and temperature stabilization of the new photometer plus the use of nightly extinction coefficients whenever available improved the external precision to between 0.003 and 0.004 mag. This is only slightly larger than the internal precision of the observations, in spite of the 1.0 mag color difference between these two stars.
Examples of observations over different timescales with this telescope are shown in Figures 11 - 13. Figure 11 displays a light curve constructed from three separate nights of data for the 12-hour secondary eclipse of RS CVn. Every 4.8 days, the F6 IV primary star in RS CVn eclipses the spotted G8 IV secondary. Eaton et al. (1993) used the slight asymmetry in the light-curve to map thedistribution of spots on the G8 star. Two 77-day rotation cycles of the spotted G5 giant AY Ceti are shown in Figure 12. As this star rotates, large starspots are carried into and out of view, resulting in the observed light variations. Tidal forces in the SB1 binary system sustain the rotation speed necessary to generate these large spots via a strong magnetic dynamo. On the second rotation, a secondary minimum appeared that revealed the emergence of a new starspot on the opposite hemisphere of the star from the spot causing the deep minimum. A 17-year light curve of the spotted star Lambda Andromedae is shown in Figure 13 . The last 12 years of data were taken with two of the automatic telescopes on Mt. Hopkins. The vertical spread in each yearly data group is caused by light variations due to rotation of starspots. The long-term variation in mean brightness suggests a starspot cycle of 11 years (Henry et al. 1995), remarkably similar to the 11-year sunspot cycle.
4. The Smithsonian/Tennessee State University 30-inch APT
The desire to search for slight luminosity variations in solar-type stars as they go through their magnetic cycles required a further improvement in the precision of robotic telescopes. We know from space-based observations that the total solar irradiance varies by 0.001 mag in phase with the sunspot cycle (Fröhlich et al. 1991), although, historically, the Sun may have undergone somewhat larger brightness excursions (Baliunas & Jastrow 1990). The measurement of such small changes in other stars from ground-based APT's requires larger telescopes, longer integrations, extremely stable photometers, carefully planned observing strategies, and scrupulous quality-control measures.
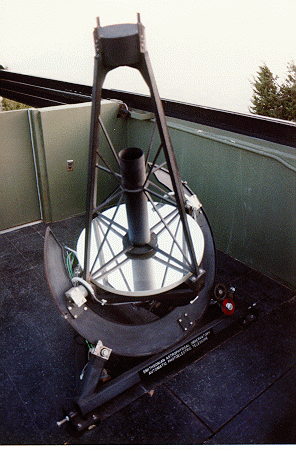
Figure 14. The Smithsonian/Tennessee State 30-inch APT. Techniques for millimagnitude photometry of solar-type stars were developed on this telescope.
The SAO/TSU 30-inch APT (Figure 14) represents a collaborative effort between the Center for Astrophysics, the Mt. Wilson Institute, and TSU to measure these small luminosity changes in solar-type stars and to correlate them with contemporaneous H & K measurements from Mt. Wilson (Baliunas et al. 1995). The 30-inch APT was constructed by Rettig Machine Shop of Redlands, California from a design by Boyd. Star Instruments produced the optics, and Boyd built the control system and precision photometer, a duplicate of the one operating successfully on the 16-inch APT. A year of development and testing finally culminated in April 1993 with the initiation of high-precision observations. Since then, over 11,000 group observations of solar-type stars have been accumulated.
The observations are made in Strömgren b and y passbands in aquartet group sequence: A,B,C,D,A,B,C,D,A,B,C,D. The use of three comparison stars (A,B,C) per program star (D) increases our chances of finding a comparison star constant to 0.001 mag or better. Sky observations were initially made at a single, central location for each group (as for the smaller APT's) after each D observation, but small systematic errors caused by gradients in sky brightness across the group when the moon was up caused us to revise the group sequence to make sky observations for each star. Standard star observations are made and reduced each night, and nightly extinction and long-term transformation coefficients are applied in the differential reductions of the program star data, just as for the 16-inch telescope. On the best photometric nights, the internal precision ranges from about 0.0010 to 0.0015 mag, compared to 0.0013 mag predicted for the sum of photon and scintillation noise. Therefore, like the 16-inch APT since its precision photometer upgrade, the 30-inch operates at the theoretical limit of internal precision for its aperture and the integration times used. The data are cloud filtered at 0.005 mag (3-sigma). However, since the highest possible precision is desired for observing solar-type stars, only data on demonstrably photometric nights (i.e. nights on which the standard star observations give a good solution for all coefficients) are used in further analysis. These selected data, therefore, have not only survived the 0.005 mag cloud filter but have all been reduced with well-determined nightly extinction coefficients.
In addition to standard star observations, other quality control observations are scheduled automatically each night to verify that all components of the telescope/photometer system are working properly. One of the most useful of these checks is the Fabry scan. Originally designed to be executed occasionally to verify that the Fabry lens was properly positioned, this test also proved useful for detecting problems with focus, CCD centering, optical alignment, filter wheel vignetting, and telescope drive systems. To run a Fabry scan, the telescope centers a star and then places it just outside the diaphragm. The telescope then steps the star through the center of the diaphragm in right ascension while the photometer takes an integration at each step. The star is recentered, and a similar declination scan is performed. The results of three such scans taken on different nights are illustrated in Figure 15. The top panel shows that the telescope is focused, that it is centering stars properly in the diaphragm, and that the Fabry lens is positioned properly to give a flat signal across the diaphragm. The middle panel indicates that a focus adjustment is needed since the wings of the scan are broadened. The bottom panel reveals that the star was not precisely centered in the diaphragm because the two scansare slightly offset from each other horizontally. Besides the Fabry scan, other tests are run each night to determine the deadtime of the photometer and to calibrate the neutral density filters (and thus to confirm that the filter wheels are functioning properly). All the standard star and quality control observations take less than 10% of the available observing time each night, and so they provide a detailed check on the quality of the night and the operation of the equipment at very low overhead.
The external precision of the 30-inch APT, as compared to the 10-inch and 16-inch telescopes, is shown in Figure 16 . In all three panels, differential V magnitudes of the constant star pair HD 125451 / HD 124570 are plotted at the same scale for a recent observing season in which all three telescopes made observations of the constant pair. The external precision of the 30-inch APT is 0.0011 mag, an order of magnitude improvement over the early APT's. Furthermore, the external errors in typical group observations for the 30-inch are the same as the mean internal errors. Thus, the temperature stabilization of the photometer and the use of nightly extinction coefficients have succeeded in minimizing the external errors.
To demonstrate the capabilities of the 30-inch APT operating at this level of precision, we plot three rotation cycles of the G7 V star HD 152391 in Figure 17, phased together on its 11.5-day rotation period (determined from this photometry). This star exhibits a clear magnetic cycle of 10.9 years as deduced from its Ca II H & K emission (Baliunas et al. 1995). Even though the amplitude of its light variability over a rotation is only 3%, the high precision of the 30-inch APT data still produces a clean light curve.
Seasonal mean magnitudes of our solar-type stars will be searched for evidence of long-term luminosity changes associated with magnetic cycles. Because roughly 50 APT group observations are obtained per star per season, each with an external precision of approximately 0.0015 mag, the formal uncertainties of the seasonal mean magnitudes will be around 0.0002 mag. Table 1 presents 1993 and 1994 seasonal means in the combined Strömgren b + y passbands for the old G1 V star HD 126053. The three comparison stars (A,B,C) appear to be extremely stable since the C-A, C-B, and B-A differential magnitudes of these comparisons all agree from one season to the next to within 0.0001 mag. The program star differential magnitudes with respect to the three comparison stars (D-A, D-B, and D-C) all show an increase in the brightness of HD 126053 (star D) of 0.0002 or 0.0003 mag from1993 to 1994. This small amplitude is just the amount the Sun's brightness would be expected to change over one year as it progresses through its magnetic cycle. With sufficiently stable comparison stars (admittedly not easy to find), it appears that we can measure these small luminosity changes in solar-type stars.
5. The Tennessee State University/Smithsonian 32-inch APT
A fourth telescope operated by TSU will go into operation at Fairborn in 1995. This 32-inch APT (Figure 18) will be dedicated to the measurement of luminosity changes in main-sequence stars that are very close to the Sun in both mass and age, a sub-group of the solar-type stars we call the solar duplicates. Construction of this telescope was originally contracted to AutoScope Corporation for location on Mt. Wilson. However, the failure of AutoScope to produce a working telescope (Henry 1995) forced us to relocate the telescope at Fairborn and have Boyd bring it into operation.
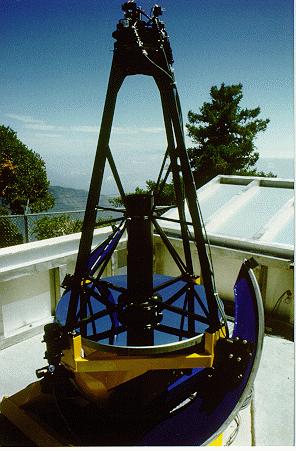
Figure 18. The Tennessee State/Smithsonian 32-inch APT. A new two-channel photometer designed for this telescope will make sub-millimagnitude observations of solar duplicates.
Because of the extremely small amplitude of the expected light variations of solar duplicates, Boyd has designed and constructed a two-channel precision photometer especially tailored for our requirements. A dichroic filter separates the Strömgren b and y passbands so that separate EMI 9124 QB photomultiplier tubes can measure the two colors simultaneously. Because scintillation noise is the principal source of error in the 30-inch telescope, we expect to improve the precision further with this telescope by doubling all integration times. The two-channel photometer allows us to do this with no loss of observing efficiency. We anticipate that the precision of a nightly group observation will be less than 0.001 mag.
Acknowledgments. It is a pleasure to acknowledge scientific collaborators Douglas S. Hall, Sallie L. Baliunas, Joel A. Eaton, and Frank C. Fekel for their part in the development of precision robotic photometry. This effort has been driven by stimulating scientific programs with unquenchable needs for more, better, and cheaper photometry. Thanks are also due to Irwin Shapiro, director of the Center for Astrophysics, for his continued support of the Fairborn Observatory site on Mt. Hopkins and to Mike Busby, director of the Center of Excellence in Information Systems, for providing a supportive environment for this work over the past several years. Of course, none of this would have been possible without the tireless expertise of Lou Boyd at Fairborn. These programs have also been supported for several years at Tennessee State University by theNational Aeronautics and Space Administration and by the National Science Foundation, most recently through NASA grant NAG8-1014 and NSF grant HRD-9104484.
References
Baliunas, S. L. et al. 1995, ApJ, 438, 269
Baliunas, S. L. & Jastrow, R. 1990, Nature, 348, 520
Boyd, L. J., Genet, R. M., & Hall, D. S. 1984, IAPPP Comm. No. 15, 20
Cristian, V.-C., Donahue, R. A., Soon, W. H., Baliunas, S. L., & Henry, G. W. 1995, PASP, 107, 411
Eaton, J. A., Henry, G. W., Bell, C., & Okorogu, A. 1993, AJ, 106, 1181
Fröhlich, C., Foukal, P. V., Hickey, J. R., Hudson, H. S., & Willson, R. C. 1991, in The Sun in Time, eds. C. P. Sonett, M. S. Giampapa, and M. S. Matthews (Tucson: University of Arizona Press), p. 11
Genet, R. M., & Hayes, D. S. 1989, Robotic Observatories, (Mesa: AutoScope)
Hall, D. S. & Henry, G. W. 1993, in Stellar Photometry - Current Techniques and Future Developments, eds. C. J. Butler & I. Elliott (Great Britain: Cambridge University Press), p. 205
Henry, G. W. 1995, IAPPP Comm. No. 57, 74
Henry, G. W., Eaton, J. A., Hamer, J., & Hall, D. S. 1995, ApJS, 97, 513
Young, A. T. 1974, in Methods of Experimental Physics, Vol. 12A (Astrophysics, Optical and Infrared), ed. N. Carleton (New York: Academic Press), p. 101
Young, A. T. 1991, private communication
Young, A. T., Genet, R. M., Boyd, L. J., Borucki, W. J., Lockwood, G. W., Henry, G. W., Hall, D. S., Smith, D. P., Baliunas, S. L., Donahue, R., & Epand, D. H. 1991, PASP, 103, 221